Ashley L. Hollingsa,b and Mark J. Hacketta,b
aCurtin Health Innovation Research Institute, Curtin University, Perth, WA 6102, Australia
bSchool of Molecular and Life Sciences, Curtin University, Perth, WA 6845, Australia
DOI: https://doi.org/10.1255/sew.2022.a11
© 2022 The Authors
Published under a Creative Commons BY-NC licence
The hippocampus (Figure 1A) is a brain region critical to spatial learning and memory. The hippocampal formation contains a highly organised architecture of neurons and neuron–neuron connections, which have been intensively studied by neuroscientists for many decades. In fact, the hippocampus is often referred to as the “Rosetta Stone” of neuroscience, with many believing elucidation of hippocampus circuitry and cell function will unravel the inner workings of the brain.
A fascinating fact of the hippocampus is that it appears to be relatively enriched in transition metal ions, particularly Fe, Cu and Zn (Figure 1B). The Zn enrichment was discovered by scientists developing histochemical methods to detect labile metals in brain tissue (e.g., works of Danscher and others),1,2 with work led by Frederickson definitively demonstrating that the characteristic pool of labile metal ions observed in the hippocampus was Zn.2–4 Of great interest, experiments aimed at depleting the labile Zn pool in the hippocampus subsequently revealed behavioural and cognitive deficits in mice,2 consistent with facets of memory loss observed during neurodegenerative diseases of ageing, such as Alzheimer’s disease.5 Consequently, a plethora of lines of research enquiries emerged, aiming to uncover the physiological and chemical pathways through which transition metal ions might be implicated in healthy memory function, and also memory loss.
Figure 1. (A) Haematoxylin and eosin histology of the hippocampus showing the characteristic organisation of brain cells (purple dots). (B) XRF elemental maps highlighting two key sub-regions of the hippocampus, the Fe-enriched neuron layer Corpus Ammonis 1 (CA1) and the Zn enriched mossy fibre region (MF) that contains numerous neuron–neuron connections (synapses). The medial to lateral orientation of the tissue is shown. Scale bar = 500 µm. Data was collected at the X-ray fluorescence microscopy beamline at the Australian Synchrotron, and is adapted with permission from Reference 12.
While the classical Timm’s histochemical stain has been invaluable to study labile Zn in the hippocampus (and brain in general), a number of important advances in this field have now been made using direct spectroscopic mapping. Specifically, the provision of intense (bright) and tuneable X-ray sources at synchrotron facilities has revolutionised the biological applications of X-ray techniques, especially X-ray fluorescence spectroscopy (XRF) and X-ray absorption spectroscopy (XAS). Key advantages of XRF are its ability to simultaneously and directly detect (map) elemental distribution at cellular resolution (and sometimes sub-cellular resolution), in situ. The direct in situ detection capabilities of XRF are critical when studying brain tissue, as chemical fixation and/or addition of staining reagents, which are common place in other microscopies, are now known to drastically alter the metal ion content and distribution within brain tissue (Figure 2).6–8
Figure 2. XRF elemental mapping of Zn distribution in (A) non-fixed flash frozen hippocampal tissue, and (B) formalin-fixed sucrose cryo-protected tissue. A substantial redistribution of Zn is observed as a consequence of formalin fixation and sucrose cryo-protection. Specifically, Zn is lost from the mossy fibres (MF, neuron–neuron connections) and redistributed to white matter tissue (WM) adjacent to the brain lateral ventricles (LV). Scale bar = 100 µm. Images adapted with permission from Reference 7.
Several important early examples of the use of XRF to study brain tissue include a definitive demonstration that the Timm’s histochemical stain reveals the presence of a labile pool of hippocampal Zn4 and the observations that transition metal ions co-localise with amyloid-β plaques (a hallmark of Alzheimer’s disease).9 More recent studies have highlighted that while amyloid-β plaques within the hippocampus appear to become enriched with metal ions in models of Alzheimer’s disease (e.g., Zn), the brain tissue surrounding the plaque becomes metal deficient.10 This has then raised an interesting research question, does metal accumulation in plaques contribute to disease pathology, or is metal deficiency a contributing factor, or both?
In addition to mapping metal ions associated with disease states, synchrotron XRF has been applied to characterise metal ion distribution in the healthy hippocampus, identifying hippocampal sub-regions locally enriched in Fe, Cu or Zn (Figure 1).11,12 Intriguingly, XRF revealed that the healthy rodent hippocampus contains an especially high Fe content within a region of hippocampal neurons (the “CA1” sector) known to display high vulnerability to neurodegeneration (e.g., neurodegenerative disease, stroke, brain trauma). Even more fascinating, the neurons within the CA1 sector that are closest to the middle of the brain (medial) contain more Fe than the neurons closer to the outside of the brain (lateral).11 The lateral-to-medial trend of increasing Fe content matches the pattern of neurodegeneration seen within this highly vulnerable brain region (i.e., medial CA1 neurons are more vulnerable than lateral CA1 neurons). Not surprisingly, a number of stroke research groups are actively using synchrotron XRF to study the relationship between Fe and neurodegeneration after stroke.13
Building from elemental mapping, a rapidly developing application of XRF and XAS beamlines at synchrotron facilities is the in situ study of metal ion speciation (oxidation state, coordination geometry, types of ligands). Continued advances at 3rd and 4th generation synchrotron facilities, combined with improvements in X-ray optics, detectors and electronics makes it now possible to collect hundreds, or even thousands, of micro-XAS spectra, to map metal speciation within biological samples. Metal speciation is most commonly studied by using a small region of the XAS spectrum, known as X-ray Absorption Near-Edge Structure (XANES). Initial applications of XANES spectroscopic mapping of biological systems include mapping the distribution of different oxidation states of S, Se and As compounds in plant tissue.14,15 The methods have now been adapted to map Fe and Cu speciation in Caenorhabditis elegans,16,17 Fe speciation in brain tissue18 and a Zn method development is in progress (Figure 3).19
Figure 3. Development of XANES spectroscopic mapping to study Zn speciation in the hippocampus. (A) XANES spectra from standard solutions of Zn2+ in the presence of different biological ligands, showing the characteristic “richness” of the XANES spectral region to differences in coordination environment. (B, C) Hippocampal Fe and Zn distribution revealing the locations of the MFs (neuron–neuron connections) and the adjacent neuron layer (pyramidal cells). (D, E) Micro-XANES spectra from the MF layer (D) and the pyramidal neurons (E) highlighting spectroscopic differences indicative of the different chemical forms of Zn present in each region. Scale bar = 50 µm. Figure reproduced with permission from Reference 19.
A number of analytical challenges still need to be resolved for XANES-mapping of brain tissue to reach its full potential. These challenges include the development of suitable spectral libraries that adequately model the different chemical forms of metal ions found in the brain, in addition to understanding the effects of sample preparation on metal ion speciation. At this stage, a great deal of work has been done by this community to optimise sample preparation to preserve elemental distribution in brain tissue, but much less is known about how oxidation state and coordination environmental of metal ions might be changing in brain tissue during the various stages of sample preparation. While challenges still exist, continued advancement in XRF and XANES spectroscopic mapping of metal ions in biological systems, and especially the brain, is an exciting prospect. Indeed, there is likely much yet to be learned about the specific role that Fe, Cu and Zn hold in supporting healthy hippocampal memory function, and how the loss of metal homeostasis could contribute to loss of memory and cognitive decline.
References
- G. Danscher and J. Zimmer, “An improved timm sulphide silver method for light and electron microscopic localization of heavy metals in biological tissues”, Histochemistry 55, 27–40 (1978). https://doi.org/10.1007/BF00496691
- R.E. Frederickson, C.J. Frederickson and G. Danscher, “In situ binding of bouton zinc reversibly disrupts performance on a spatial memory task”, Behav. Brain Res. 38(1), 25–33 (1990). https://doi.org/10.1016/0166-4328(90)90021-6
- C.J. Frederickson, B.A. Rampy, S. Reamy-Rampy and G.A. Howell, “Distribution of histochemically reactive zinc in the forebrain of the rat”, J. Chem. Neuroanat. 5(6), 521–530 (1992). https://doi.org/10.1016/0891-0618(92)90007-D
- D.H. Linkous, J.M. Flinn, J.Y. Koh, A. Lanzirotti, P.M. Bertsch, B.F. Jones, L.J. Giblin and C.J. Frederickson, “Evidence that the ZNT3 protein controls the total amount of elemental zinc in synaptic vesicles”, J. Histochem. Cytochem. 56(1), 3–6 (2008). https://doi.org/10.1369/jhc.6A7035.2007
- P.A. Adlard, J.M. Parncutt, D.I. Finkelstein and A.I. Bush, “Cognitive loss in zinc transporter-3 knock-out mice: a phenocopy for the synaptic and memory deficits of Alzheimer’s Disease?”, J. Neurosci. 30(5), 1631–16363 (2010). https://doi.org/10.1523/JNEUROSCI.5255-09.2010
- M.J. Hackett, J.A. McQuillan, F. El-Assaad, J.B. Aitken, A. Levina, D.D. Cohen, R. Siegele, E.A. Carter, G.E. Grau, N.H. Hunt and P.A. Lay, “Chemical alterations to murine brain tissue induced by formalin fixation: implications for biospectroscopic imaging and mapping studies of disease pathogenesis”, Analyst 136, 2941–295 (2011). https://doi.org/10.1039/C0AN00269K
- M.J. Pushie, A. Hollings, J. Reinhardt, S.M. Webb, V. Lam, R. Takechi, J.C. Mamo, P.G. Paterson, M.E. Kelly, G.N. George, I.J. Pickering and M.J. Hackett, “Sample preparation with sucrose cryoprotection dramatically alters Zn distribution in the rodent hippocampus, as revealed by elemental mapping”, J. Anal. At. Spectrom. 35, 2498–2508 (2020). https://doi.org/10.1039/D0JA00323A
- J. Chwiej, M. Szczerbowska-Boruchowska, M. Lankosz, S. Wojcik, G. Falkenberg, Z. Stegowski and Z. Setkowicz, “Preparation of tissue samples for X-ray fluorescence microscopy”, Spectrochim. Acta B 60(12), 1531–1537 (2005). https://doi.org/10.1016/j.sab.2005.10.002
- L.M. Miller, Q. Wang, T.P. Telivala, R.J. Smith, A. Lanzirotti and J. Miklossy, “Synchrotron-based infrared and X-ray imaging shows focalized accumulation of Cu and Zn co-localized with β-amyloid deposits in Alzheimer’s disease”, J. Struct. Biol. 155(1), 30–37 (2006). https://doi.org/10.1016/j.jsb.2005.09.004
- S.A. James, Q.I. Churches, M.D. de Jonge, I.E. Birchall, V. Streltsov, G. McColl, P.A. Adlard and D.J. Hare, “Iron, copper, and zinc concentration in Aβ plaques in the APP/PS1 mouse model of Alzheimer’s Disease correlates with metal levels in the surrounding neuropil”, ACS Chem. Neurosci. 8(3), 629–637 (2017). https://doi.org/10.1021/acschemneuro.6b00362
- M.J. Hackett, A. Hollings, S. Caine, B.E. Bewer, M. Alaverdashvili, R. Takechi, J.C.L. Mamo, M.W.M. Jones, M.D. de Jonge, P.G. Paterson, I.J. Pickering and G.N. George, “Elemental characterisation of the pyramidal neuron layer within the rat and mouse hippocampus”, Metallomics 11(1), 151–165 (2019). https://doi.org/10.1039/c8mt00230d
- N. Fimognari, A. Hollings, V. Lam, R.J. Tidy, C.M. Kewish, M.A. Albrecht, R. Takechi, J.C.L. Mamo and M.J. Hackett, “Biospectroscopic imaging provides evidence of hippocampal Zn deficiency and decreased lipid unsaturation in an accelerated aging mouse model”, ACS Chem. Neurosci. 9(11), 2774–2785 (2018). https://doi.org/10.1021/acschemneuro.8b00193
- M.J. Pushie, N.J. Sylvain, H. Hou, S. Caine, M.J. Hackett and M.E. Kelly, “Tracking elemental changes in an ischemic stroke model with X-ray fluorescence imaging”, Sci. Rep. 10, 17868 (2020). https://doi.org/10.1038/s41598-020-74698-2
- I.J. Pickering, E. Yu Sneeden, R.C. Prince, E. Block, H.H. Harris, G. Hirsch and G.N. George, “Localizing the chemical forms of sulfur in vivo using X-ray fluorescence spectroscopic imaging: application to onion (Allium cepa) tissues”, Biochemistry 48(29), 6846–6853 (2009). https://doi.org/10.1021/bi900368x
- I.J. Pickering, C. Wright, B. Bubner, D. Ellis, M.W. Persans, E.Y. Yu, G.N. George, R.C. Prince and D.E. Salt, “Chemical form and distribution of selenium and sulfur in the selenium hyperaccumulator Astragalus bisulcatus”, Plant Physiol. 131(3), 1460–1467 (2003). https://doi.org/10.1104/pp.014787
- S.A. James, R. Burke, D.L. Howard, K.M. Spiers, D.J. Paterson, S. Murphy, G. Ramm, R. Kirkham, C.G. Ryanc and M.D. de Jonge, “Visualising coordination chemistry: fluorescence X-ray absorption near edge structure tomography”, Chem. Commun. 52, 11834–11837 (2016). https://doi.org/10.1039/C6CC06747F
- S.A. James, B.R. Roberts, D.J. Hare, M.D. de Jonge, I.E. Birchall, N.L. Jenkins, R.A. Cherny, A.I. Bush and G. McColl, “Direct in vivo imaging of ferrous iron dyshomeostasis in ageing Caenorhabditis elegans”, Chem. Sci. 6, 2952–2962 (2015). https://doi.org/10.1039/C5SC00233H
- M.J. Hackett, G. Ellison, A. Hollings, F. Colbourne, M.D. de Jonge and D.L. Howard, “’A spectroscopic picture paints 1000 words’ mapping iron speciation in brain tissue with ‘full spectrum per pixel’ X-ray absorption near-edge structure spectroscopy”, Clin. Spectrosc. 3, 100017 (2021). https://doi.org/10.1016/j.clispe.2021.100017
- A.L. Hollings, V. Lam, R. Takechi, J.C.L. Mamo, J. Reinhardt, M.D. de Jonge, P. Kappen and M.J. Hackett, “Revealing differences in the chemical form of zinc in brain tissue using K-edge X-ray absorption near-edge structure spectroscopy”, Metallomics 12(12), 2134–2144 (2020). https://doi.org/10.1039/d0mt00198h
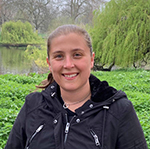
Ashley Hollings
Ashley Hollings is completing her PhD at Curtin University under the supervision of Dr Mark Hackett, sitting at the interface of analytical chemistry and neuroscience, specifically focussed on developing new spectroscopic protocols to image brain metals and biochemistry during ageing. Ashley’s PhD incorporates a multi-modal imaging approach, using X-ray absorption near edge structure (XANES) spectroscopy alongside X-ray Fluorescence Microscopy (XFM), synchrotron radiation Fourier Transform Infrared (SR-FT-IR) microscopy and confocal Raman microscopy. Ashley hopes that the combination of X-ray spectroscopy and vibrational spectroscopy will help to reveal links between metal dis-homeostasis and altered brain biochemistry during ageing and dementia.
ashley.hollings@postgrad.curtin.edu.au https://orcid.org/0000-0001-7829-4932
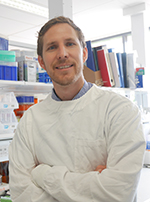
Mark Hackett
mark.j.hackett@curtin.edu.au
